The β‐sheet forms as a result of layering of the protein chain, one part on top of the other. This structure is stabilized by hydrogen bonds between neighboring parts of the chain ( Figure 2.21). These β‐sheets can form in two ways, either from neighboring protein chains that run in the same orientation (called parallel chains) or from an individual protein chain that runs back and forth (antiparallel chains). This arrangement of the chain backbone along with hydrogen bonding results in both types of β‐sheet having a rather rigid structure. The side groups are at right angles to the sheets, minimizing steric hindrance between the layers. Regions of β‐sheets are common in many proteins because of their efficient packing.
Figure 2.21 Illustration of β‐sheet structure generated by layering of polypeptide chains and hydrogen bonding between the chains: (a) parallel β‐sheet, (b) antiparallel β‐sheet, and (c) β‐turn in antiparallel β‐sheet.
In forming an antiparallel β‐sheet, the chain backbone of the protein changes its direction, turning back and forth upon itself, making what is described as a β‐turn. This is an important feature of protein folding, which gives a more compact shape. A hydrogen bond between the first and third peptide residues of the β‐turn and the amino acid proline are frequently found at bends in the chain backbone ( Figure 2.21c). Of the 20 α‐amino acids ( Figure 2.17), proline is the only one in which the side chain is connected to the chain backbone twice, forming a five‐membered nitrogen‐containing ring. Because ring structures typically have limited flexibility, proline is unable to occupy many of the main‐chain conformations easily adopted by other amino acids. Proline is also nonpolar and, thus, cannot take part in hydrogen bonding. Together, these factors make proline a useful residue for occupying tight turns in a protein structure such as the β‐turn.
The tertiary structure of a protein refers to the overall three‐dimensional shape (conformation) of its polypeptide chain. Many proteins do not have the regular structure of the α‐helix or β‐sheet but, instead, rely for their properties on their tertiary structure. A polypeptide chain can fold in a complex manner to give a variety of three‐dimensional shapes, but two broad classes of structures, referred to as globular and fibrous, are often distinguished. In globular proteins, the polypeptide chain is more compactly folded like a ball of string with an irregular surface. In comparison, the polypeptide chain in fibrous proteins have a more elongated or filamentous three‐dimensional structure ( Figure 2.22). A fibrous protein often has a length that spans a longer distance than the average diameter of a globular protein.
Figure 2.22 Schematic illustration in two dimensions of the overall three‐dimensional shape of (a) globular proteins and (b) fibrous proteins.
In globular proteins, atoms in the side groups attached to the chain backbone can form covalent bonds and noncovalent bonds, such as ionic, hydrogen and van der Waals bonds, with atoms of other side groups. Unlike hydrogen bonding in the secondary structure that involves the amide bond in the chain backbone without involving the side chains, these bonds can involve side groups of the same chain (intrachain bonding) as well as different chains (interchain bonding). As a result of these interactions, the chain can fold up into various complex three‐dimensional shapes that include lengths of randomly coiled chain along with regions having the regular repeating pattern of the β‐sheet or α‐helix.
Of the approximately twenty naturally occurring α‐amino acids, only one, cysteine, offers the possibility of forming a covalent bond while approximately five have side groups than can form ionic bonds ( Figure 2.17). Consequently, hydrogen and van der Waals bonds often have the largest effect in determining the tertiary structure of a protein. Although these bonds are much weaker than the covalent and ionic bonds ( Table 2.2), the combined effect resulting from a large number of such noncovalent bonds determines and stabilizes the globular three‐dimensional shape of the protein. Each type of protein has its own particular conformation but the conformation adopted by any protein is typically the one with the minimum free energy.
Examples of the main types of interactions between the side groups that can determine the conformation of a protein chain are:
Covalent bonds: Two residues of the amino acid cysteine, for example, can be oxidized at their thiol (S–H) groups to form a disulfide (S–S) bond, sometimes called a disulfide bridge ( Figure 2.23a). Reduction reverses this process.
Ionic bonds: The side groups of some amino acid residues ( Figure 2.17) contain weakly acidic or weakly basic groups that are deprotonated or protonated at the physiological pH (~7.4), giving rise to atoms in these molecules that have a negative or positive charge, respectively. Electrostatic attraction between oppositely charged atoms results in an ionic bond ( Figure 2.23b).
Figure 2.23 Illustration of the main types of interactions between side groups of proteins which can determine the conformation of a protein, using examples of specific amino acid residues. (a) Covalent bond between cysteine (Cys) residues; (b) ionic bond between aspartic acid (Asp) and lysine (Lys) residues; (c) hydrogen bond between serine (Ser) residues; (d) van der Waals bonding between leucine (Leu) and valine (Val) residues.
A common group is the carboxyl group (C=O)OH in which the H atom dissociates to give a (C=O)O −species with a negatively charged oxygen atom. This group is found in the side groups of the negatively charged amino acids ( Figure 2.17). On the other hand, weakly basic groups are composed of nitrogen‐containing species such as the amine (NH 2) group. These groups are found in the side groups of the positively charged amino acids. The nitrogen atom in these species become protonated to form a positively charged nitrogen atom.
It should be noted that in these weakly acidic or basic groups, not all of the (C=O)OH or nitrogen‐containing groups become deprotonated or protonated. Instead, there is an equilibrium reaction that can be expressed generally by the equations
(2.8) 
(2.9) 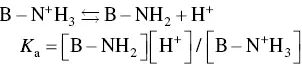
where A–COOH and B–NH 2represent the side groups, the square brackets denote the concentration of the respective species, and K ais the equilibrium constant for the reaction. A well‐known indicator of the acidity or basicity of these weak acids or bases is their p K avalue, defined as
(2.10) 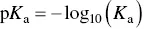
Читать дальше