Some repressors and activators present in bacteria can also be found in bacteriophages , viruses that attack bacteria. Bacteriophages, or phages for short, are essentially made of DNA and a few proteins packaged within an outer coat of proteins. The protein cl is a major player in the life of the phage lambda.
Phages, such as lambda phage, can be lytic or lysogenic. A phage is lytic if it causes lysis (from the Greek lysein , meaning to dissolve) of the infected host cell. Lysis occurs when a phage injects its DNA into a bacterium. The DNA circularizes and proceeds to replicate into new bacteriophages until the sheer number of them makes the bacterium explode. In contrast, a phage is lysogenic if the phage's DNA, injected into the bacterium, becomes integrated into the host bacterial cell's chromosome and becomes silent. In some conditions, it can excise and again act as a lytic phage.
The cI protein is a regulator at the heart of both the lytic and lysogenic situations in the lambda phage. cI is able to behave like a repressor when the phage DNA integrates itself into the bacterial genome. cI inhibits the expression of bacterial excision genes that allow the phage DNA to excise from the bacterial chromosome. If bacteria are stressed, irradiated, or grown in certain nutrient deficiencies, the bacterial protein RecA is activated. It cleaves the cI protein, allowing excision to occur.
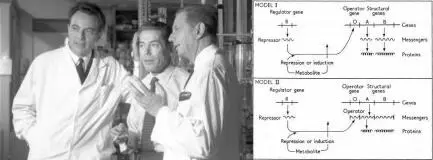
Figure 6. (Left) The three 1965 Nobel Prize winners François Jacob, Jacques Monod, and André Lwoff. (Right) Jacob, Monod, and Lwoff's famous model proposing two possible alternatives for a simultaneous repression of the three genes in the lactose operon. Note that RNA here plays the role of the repressor.
However, in the beginning of the 1980s, it was found that the replication of plasmids—small circular minichromosomes that carry certain accessory genes, such as those for virulence or antibiotic resistance—is controlled by small RNAs that attach (or hybridize) to single strands of the plasmid’s DNA and prevent the plasmid’s replication. The concept of antisense RNA was thus born and the RNA revolution began.
Several other antisense RNAs were then discovered in bacteria, but no one could have foreseen the explosion of knowledge in the early 2000s following the discovery of microRNAs in eukaryotes. MicroRNAs are small RNAs with 22 nucleotides that attach to the 3' region of eukaryotic RNA and affect its translation. This surge of new discoveries was made possible by revolutionary new technologies, in particular the DNA chips called tiling arrays and new ultrarapid sequencing methods for genomes and RNA. These techniques allowed for the analysis of the ensemble of RNA transcripts of bacteria grown under a variety of conditions and led to the discovery that bacteria express a large number of RNA transcripts that do not encode proteins. These transcripts, called noncoding RNAs , are often the product of the intergenic regions of DNA and can act as regulators. Bacteria can have as many as several hundred distinct noncoding RNAs.
Many RNAs originally categorized as noncoding RNAs or as regulatory RNAs do regulate gene expression themselves. They can also code for small peptides. This is the case for RNAIII of Staphylococcus aureus .
While investigating the noncoding RNA in the bacterium Streptococcus pyogenes , the research group led by Emmanuelle Charpentier in 2010 made an extremely important discovery. They demonstrated that a small RNA known as tracrRNA , or trans-activating CRISPR RNA, plays a critical role in how the CRISPR system (for clustered regularly interspaced short palindromic repeats) recognizes invading phages and, more specifically, is involved in the destruction of these phages. This research has led to spectacular and unexpected developments, in particular the revolutionary technology called the CRISPR/Cas9 technology for the modification of genomes, as described in the next chapter.
Noncoding RNAs vary widely in size, ranging from tens to hundreds or even thousands of nucleotides. They can interact quite efficiently with other RNAs or even with DNA, as well as with some proteins. They can sometimes act as antisense RNA, even if the noncoding RNA and its target are not entirely complementary. They often prevent mRNA translation by attaching themselves to the translation initiation site. But they can also stimulate translation by attaching to the region of the RNA situated upstream of the genes and changing its structure and configuration in order to stimulate gene translation—for example, by uncoiling a formerly hidden region that prevents ribosomes from accessing their site of action. Noncoding RNA can also bind to proteins, sequester them, and thus prevent them from acting. This situation is thought to be uncommon in nature, although a few examples are well documented, for instance, the CsrA protein that is sequestered by small CsrB RNA in E. coli .
Riboswitches: molecular interrupters
Some noncoding RNA elements, called riboswitches , function as interrupters. Situated at the beginning of certain mRNAs, a riboswitch can fold in two different ways depending on its binding to its specific ligand. If the riboswitch binds to a ligand, its RNA can take on a form that either impedes the translation of the mRNA (translational riboswitch )—in which case the entire mRNA is synthesized but its message is silenced—or stops the transcription of the genes downstream of it ( transcriptional riboswitch ), which leads to the synthesis of a very short RNA. If the riboswitch does not bind to the ligand, the RNA is transcribed in its entirety and is also completely translated. These riboswitch ligands vary greatly in nature, from S-adenosylmethionine (SAM) to vitamin B 1or B 12to transfer RNA or metals such as magnesium.
Riboswitches not only regulate mRNAs as explained above, they can also regulate noncoding RNA. There is such a case in the foodborne pathogen Listeria monocytogenes , in which a vitamin B 12riboswitch controls an antisense noncoding RNA for the regulator of a series of genes. These genes encode enzymes that process propanediol, a compound present in the intestine that is produced by the fermentation of certain sugars by commensal bacteria. These enzymes require vitamin B 12to function as follows: (i) in the presence of B 12, the riboswitch leads to the synthesis of a short RNA while allowing synthesis of the regulator protein PocR, and PocR then activates the synthesis of genes under its control; (ii) in the absence of vitamin B 12, the riboswitch is configured such that a long form of antisense noncoding RNA is produced, which hybridizes to the mRNA that codes for PocR, stopping its production. Thus the PocR activator is not produced unless conditions are favorable, that is, if proteins encoded by the genes that it regulates can be activated by vitamin B 12.
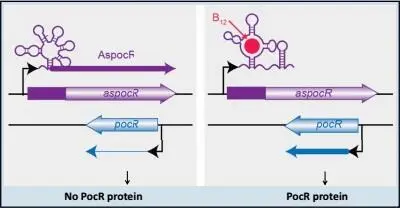
Figure 7.Schematic representation of the chromosome region encoding PocR. In the absence of vitamin B 12(left), the long transcript AspocR hybridizes with the transcript for pocR , which is then destroyed, preventing the synthesis of PocR. In the presence of B 12, the pocR messenger RNA allows synthesis of the protein PocR.
Another example of a nonclassical riboswitch is a different vitamin B 12riboswitch found in L. monocytogenes and Enterococcus faecalis , both of which cause intestinal infections. This riboswitch controls a noncoding RNA that can sequester a regulator protein that activates the eut genes. eut genes code for proteins involved in the utilization of ethanolamine, a compound found in abundance in the intestine. The riboswitch works as follows: (i) if vitamin B 12is present, a short form of RNA is produced, a form that does not sequester the regulator protein, which is then free to activate the expression of the eut genes; (ii) if vitamin B 12is absent, a long form of noncoding RNA is produced that sequesters the regulator protein, which is thus unable to activate the eut genes.
Читать дальше