and the rotor speed v as
(2.6) 
The function has a maximum with x = 1/3 with power coefficient von 16/27. This equals 59.3% of the incoming kinetic power of the theoretical limit of the electrically usable power. The Betz limit covers flow losses due to the design of the rotor blades. Additional mechanical losses are caused by fraction, the inertia of the bearing, and the converter behind the rotor, typically electromagnetic. So far, large wind turbines demonstrated a conversion efficiency of about 40%. New types of design might exceed those limits. However, microturbines are expected to reach a range of 10% due to nonlinear effects and the small ratio of converter to loss surface [13, 31].
The flow speed in HVAC plants can reach up to 6 m/s. For an air density ρ = 1.2 kgcm -3, an efficiency of 10% and an area of 1 cm², an electric power of 1.3 mW could be achieved. The current European low-pressure systems reach up to 3 m/s, with a resulting power output of approximately 0.2 mW.
Although their maximum power limit is significantly below the limits of large gas flow turbines, indoor air flow of all sources can be a very valuable power source with achievable power in the milliwatt scale. This is sufficient to power most IoT applications.
2.2.1.4 Acoustic Vibrations
Acoustic vibrations in indoor environments are available from humans, machines and outdoor vibrations, including traffic. Their harvest is one of the youngest disciplines in Micro Energy Harvesting with applications ranging from typical IoT systems, noise reduction to espionage and hacking. Typical designs are based on, but not limited to, the use of electromagnetic and piezoelectric converters or a combination of both. Yuan et al . provided a recent review of the field [32].
Most converters achieve a power in the range of a few microwatts with converters reaching the lower milliwatt scale as the exception.
Elastic energy has had a long tradition in human application, long before electricity was discovered. Watches and clocks have been built based on winding springs that stored energy in its elastic form, which then actuated the pendula and the mechanics. The first turntables and many other devices used the same principles. A common example of an elastic micro energy harvester is the Seiko Kinetic watch.
2.3 Thermoelectric Conversion
Thermal energy can be converted to electric energy with thermoelectric generators (TEGs). In their typical design, TEGs consist of semiconductor materials. Thermocouples, that is pairs of p- and n-type legs, are connected in parallel thermally and in series electrically. The main physical effects in TEGs are the Seebeck, Thompson and Peltier effect, respectively, and Joule heating.
A temperature difference ∆ Tg in such a thermocouple generates an electric potential. Following the Seebeck effect, the resulting output voltage U for N pairs of thermocouples is
(2.7) 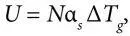
where α sdenotes the Seebeck coefficient in [V/K] of the thermocouple.
The ohmic resistance causes thermal losses of an electric current I in a conductor of a resistance R , also known as Joule heating P J
(2.8) 
In a heterogeneous material consisting of materials A and B, an electric flow at the material junction yields a heat flow. This is described by the Peltier effect, where π ABis the Peltier coefficient of the material in [V], with
(2.9) 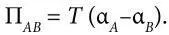
In a homogeneous material, the emission and absorption of heat due to temperature difference in a conductor is represented by the Thomson effect. The Thomson coefficient in [V/K] is
(2.10) 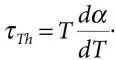
The influence of these effects on the TEG performance depends on the size, design and the application environment. Freunek et al. have investigated the detailed modeling of the performance of TEG [33].
Any temperature difference of an environment couples into the TEG with a thermal resistance K g. K gis optimized when it equals the sum of the coupling resistance of the hot and the cold T hand T cside of the TEG, respectively, where
(2.11) 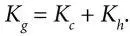
The maximum achievable temperature difference decreases with increasing miniaturization. The heat sink needs to be dimensioned in a sufficient size, to make a temperature difference usable for a thermoelectric conversion. The size of the heat sink therefore is mostly the limit to miniaturization in TEG systems.
The maximum power output of a TEG P outcan be approximated to [33]
(2.12) 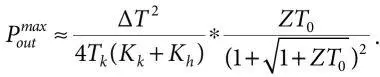
The thermoelectric figure of merit Z is device specific. Figure 2.3 provides a schematic of a TEG.
The output voltage of TEG typically ranges around a few 100 mV. Therefore, DC/DC converters are required.
In indoor industrial applications, the heat from machines can be used with the room temperature as cold sink. In these applications, and especially if the system size is not limited, TEG systems can achieve output powers in the milliwatt range. The same is valid in cold areas or seasons if the temperature difference between outside and indoors can be made usable. However, in most consumer and office applications, the main source for a temperature potential is the difference between wall temperatures and the room temperature. In this case, a TEG will yield a few microwatts.
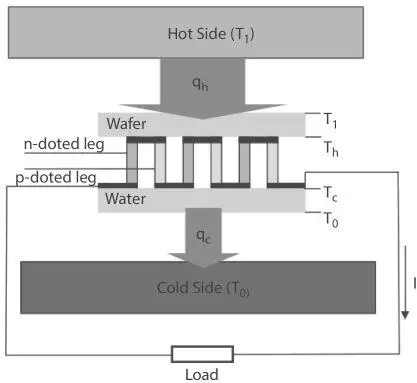
Figure 2.3Schematic of a TEG with n- and p-type legs, and a hot and cold side.
2.4 Electrochemical Potential
The electrochemical potential from biological sources can be converted to electric energy. Biologic sources have the advantage that the host continuously and actively recharges these power sources by eating and drinking or photosynthesis. Such a host for a biochemical converter can be a plant, an animal or a human.
The first research on the use of biochemical energy, such as adenosine triphosphate (ATP) in the human body, was the ATP powered nanomotor by Cornell University [34]. Voltree Power’s bioenergy harvester uses the electrochemical potential resulting from the different pH concentration between soil and a plant [35]. The output voltage was reported to reach 50 to 200 mV for an estimated short circuit current between 0.1 to 1 mA [36]. The induced voltage U for an output voltage U ʹ results from the Nernst equation
Читать дальше